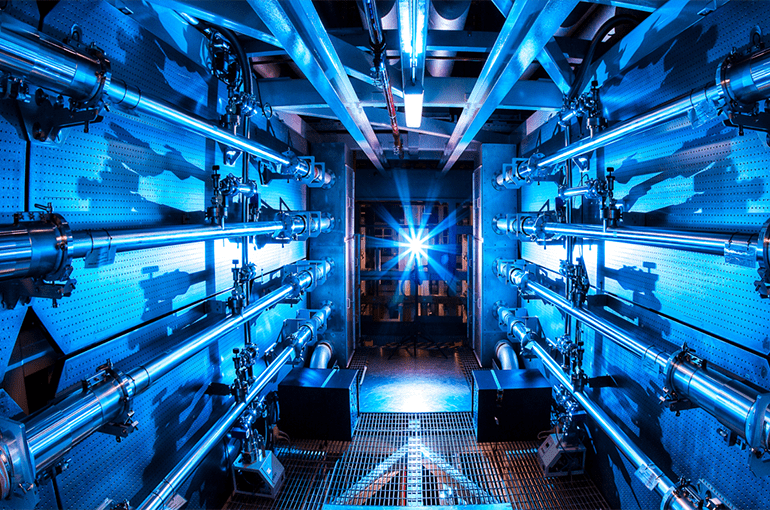
In the first part of this double article we’ve seen that achieving nuclear fusion is indeed possible. Well… at least in the lab. In this second part, as promised, we’ll take a look at the technologies which hope to take Nuclear Fusion out of the lab and into our (very real) World, where lobbies, budgets, competition, standardization and national interests have to be taken into account.
ITER – The Big, Bad Donut
TOKAMAK is the acronym for the Russian equivalent of something like “toroidal chamber with magnetic coils”. The name is actually quite self-explanatory, since this type of reactor is pretty much a donut-shaped chamber (a.k.a. torus) surrounded by huge magnets.
Although this type of reactor is being the subject of critics nowadays, its history is very relevant. It was born in Russia in the 50’s, both in concept and design, with a number of prototypes called T-1 up to T-15 (apparently USSR culture was quite straightforward when it came to naming things). Results from the T-3 (commissioned in 1962) marked a turning point in fusion research, 48 years ago, at the IAEA Novosibirsk conference.
Needless to say, the Cold War focused most of nuclear fusion research towards Weapons Design, rather than energy research. Also, the engineering was (very) hard, meaning the process of designing, creating and optimizing materials and technologies didn’t ran at our (nowadays apparent) ultra-fast pace. The TOKAMAK became the most extensively studied design, which made it the prime candidate for a large experiment when that day arrived. And it did.
Fast forward to 1985. The scenario is the Geneva Superpower summit (today’s equivalent of the G-8 except at the time was basically a G-2). The USSR’s General Secretary Gorbachev and USA’s President Ronald Reagan reach an agreement on a collaborative international project to develop fusion energy for peaceful purposes. Countries kept joining, signing, updating and renegotiating that agreement for the best part of 2 decades until, in 2006 at the Elysée Palace in Paris, the ITER Agreement took place. There and then, seven of the World’s largest countries (China, EU, India, Japan, Korea, Russia and USA) joined forces to create the first large scale prototype of a nuclear fusion reactor – the ITER (International Thermonuclear Energy Reactor).
Reaching an agreement between two people is sometimes hard. Therefore, reaching a massive international agreement to build something that was never done before to solve a problem that some (thankfully just some) still claim doesn’t exist (i.e. the “energy crisis”) has to be painstakingly hard. As we’ve seen, when the choice had to be made, the TOKAMAK was the best placed to win the honours.
Therefore, at this very moment when you are reading this, near the small 1000 people “Commune” of Saint-Paul-lès-Durance in Southern France, a 20 thousand million USD experimental facility, with which we hope to achieve the insane temperatures of roughly 150 million ºC (more or less 10 times the temperature in the Sun’s core) is being built.
Just like any other power plant, ITER hopes to turn water into steam and then use turbine generators to produce electricity. The difference is how we produce that heat. The process starts by creating a nearly perfect vacuum, in other words, it all happens inside a hermetically sealed steel container, with double walls, 19.4 metres across (outer diameter) and 11.4 metres high, weighting approximately 8500t. If you think this container is huge, this container is actually inside another chamber called the Cryostat. With 30m in width and 30m in height, this stainless steel casing weighing 3850t, will also be in a vacuum (at a pressure 1.000.000.000 times inferior to the one you are feeling right now).
Next, the magnet systems (that will help to confine and control the plasma) are charged up and the gaseous fuel is introduced. But not just any magnets: 10.000t of magnets, cooled with supercritical helium to -269 °C, built from a material with such little production (niobium-tin superconducting strands) that just because of this project, global demand for it increased 6x.
As the plasma heats up, equipment is required to remove the heat and impurities from the chamber. 440 modules (weighting a modest 4.6t each) made of beryllium, high-strength copper and stainless steel, completely cover the inner walls of the vacuum chamber (the “blanket”) to protect the steel structure and the superconducting magnets from the heat and the neutrons.
At the bottom of the vacuum vessel, lies a fundamental part of the reactor: the divertor. This is where the heat and particle bombardment will hit the hardest. The heat flux sustained by this element will be between 10-20MW/m2 (if you thing the subway in rush hour is hot, imagine 300.000 people in a square of 1m by 1m). The chemical element with the highest melting point is tungsten, 3414 °C, and that’s why it was chosen as a coating for this component.
The blanket and the divertor will be cooled with water and are designed to remove up to 736 MW of thermal power, which will then be converted to electricity.
If we’re spending this much money then for sure it’s going to work
Aside from the fact that the previous statement essentially defies the very purpose of basic research funding, it does raise an uncomfortable question: do we know enough about scaling up nuclear fusion to spend this much money (about 20 billion USD) erecting this monolith? There are some very credible arguments pointing towards the NO.
- The most obvious problem has already been approached in the first article. It’s called Tritium and it is part of the ITER fuel. We don’t have it but the worst part is: for all we know, there’s no evidence that a consistent self-sufficient tritium breeding from Lithium can actually be achieved. Which means that, pouring some extra million USD into ITER to add Lithium blankets might solve nothing (though it will for sure teach us something).
- Tritium is dangerous stuff and can be used in weapons manufacturing (although in a far more sophisticated manner than, for instance, Uranium). Even handling grams of Tritium is a challenge, so one can only imagine handling the kilograms of this substance the ITER hopes to manage.
- The materials that compose the chamber will be subject to massive wearing. We cannot reasonably assure that the materials chosen for ITER can withstand the workload at an effective cost/benefit ratio and, if they can’t, how and when can we come up with materials that do.
- And last but not least, commercial energy production will require steady state fusion conditions at least comparable to that of today’s standard nuclear fission reactors (which at this massive scale implies something at least a little under 1GW). Apart from the obvious financial break-even point question, there’s another, more fundamental, problem: even under the (constantly updated) ITER planning we’re looking at 2025 for first-plasma! Assuming nothing fails in such a complex machine (which if we take LHC as reference most likely something will), we’re looking at a very long timeframe to achieve steady fusion, in which many things can happen reducing the need for a huge TOKAMAK experiment.
How about other players?
The TOKAMAK is not the only alternative to generate fusion, there are other design proposals. Even though these have even less track record (so believing in them can be seen as a mere exercise of faith) it’s in these other technologies that the hope of many (in particular the Private sector) is deposited. Here’s a list:
Many organizations have and hope to keep pursuing this type of system, such as EMC2, ProtonBoron or RadiantMatter.
The EMC2 is an out of the ordinary organization, with a military past and a civil future, which plans to complete the final scientific proof of principle for the polywell (potential well) reactor technology around 2019-2020, and for which is currently trying to raise 30 million USD. It’s their belief that they can create a prototype commercial net energy gain fusion reactor by 2030, at a fraction of the cost of any TOKAMAK based reactor.
This reactor consists of a cubical group of stainless steel donuts, called a MaGrid, encased in a vacuum chamber. Inside each donut is a coil, which induces a magnetic field that acts as a trap to an electron cloud in the centre. This concentration of negative electric charge accelerates Boron and Hydrogen ion beams to the centre of the MaGrid. When the protons and Boron nuclei collide, a nuclear reaction occurs. The reaction creates high-energy alpha particles (helium nuclei), which carry large amounts of energy away from the centre. Notice the fuels are different from the ones mentioned before? This reaction is aneutronic: meaning no nuclear radiation is leaked. As if that was not cool enough: there’s no Tritium anywhere to be seen (which is always good news).
EMC2 claims it made a major breakthrough in polywell technology in 2013, independently verified by the US Navy. But, although the theory sounds great, there’s simply not enough information about this technology available to conclude it is even feasible at a large scale.
Unlike ITER, which is aimed at testing the production of electrical energy, the NIF is purely a research experiment. It relies on powerful laser beams to compress and heat the hydrogen isotopes to the point of fusion, a technique called inertial confinement fusion, or ICF. A laser pulse is carried onto 192 main laser amplifier beamlines and then onto two ten-story switchyards on either side of the target chamber, a trip of 1500m lasting 5 microseconds, which ends up depositing a massive 1.85 MJ of ultraviolet laser light in a little target, making it the most powerful laser on the planet.
Either the laser beams are focused “directly” on a small spherical pellet containing micrograms of deuterium and tritium or “indirectly” on the inner walls of a gold cavity (called a hohlraum) which reflect them towards the fuel pellet. In any case, the rapid heating of the outer layer of the target explodes it but, since the target is confined by the lasers, the remaining portion is driven inwards, causing further compression of the fuel and the formation of a shock wave which further heats the fuel until fusion occurs.
In 2015 this system was little over half way onto producing more energy than the one it consumes.
There are also other contestants in this race such as:
General Fusion (funded by Amazon’s CEO Jeff Bezos) with its Magnetized Target Fusion
Helion Energy (funded by Peter Thiel’s Mithril Capital) with its Magneto-Inertial Fusion
TriAlpha (funded by Microsoft’s cofounder Paul Allen) with its Colliding Beam Fusion Reactor
The final competitor in this race is actually a Country: Russia. According to some news, Russia wants to upgrade its TOKAMAK T-15 reactor in 2018 to create a fusion-fission hybrid reactor which uses the neutrons released from the fusion reaction to maintain fission reactions, ergo multiplying the energy output. This project is (apparently) “open to international collaboration” although it seems that by “international” the country actually means China.
SUMMARY – Is it worth it?
Dr. Dittmar states that, from a purely analytic point of view, the building of a TOKAMAK under ITER may amount to little more than “an expensive experiment to investigate some fundamental aspects of plasma physics”. He’s most likely not wrong.
Nevertheless, it’s important to remember that our Society seldom makes the most efficient choice. This means that the decision to build a massive TOKAMAK was only partially scientific (i.e. based on empiric evidence). Also these large endeavours test the limits of our supply and manufacturing chains, giving them the resources to update themselves and teach us a lot from the operational point of view (which can positively impact many industries). It also drives research which, just like in the previous case, can lead to very interesting results and innovations in unexpected fields.
Not convinced? Well if we learned a lesson from the Apollo Program (which could very well be called little more than “an expensive experiment to land Humans on the moon”) is that the qualitative outcomes can be as important as the quantitative ones, at least when it comes to mobilizing the sector of the population which is not inclined towards these particular questions.
But Alas! Good intentions don’t make our energy future more certain, and pouring extra millions onto ITER just because we already spent a lot may not be the way to go (this is something the reader must judge for himself).
For me, unless one of these private and secretive organizations turns out to be both right enough and lucky enough to reach a destiny-defying breakthrough, it may be several decades before we see a real advance in stable-state fusion and several more until they are compacted into a size that can really disrupt the World’s very conception of what We, as a species, can achieve when given such a massive power source. Undoubtedly we’ll get there but, until then, we have to rely on other technologies that deserve all the investment, interest and incentive we can muster (which is far more than what we are already putting in).
Hugo Martins | Analyst
If you found it interesting, please share it!
Recent Articles